REPORT
Seeking the sensational on a small scale
In a huge hall in Canton Aargau, researchers are revisiting the basic building blocks of physics. Horizons visits a world promising sensational discoveries on a tiny scale.
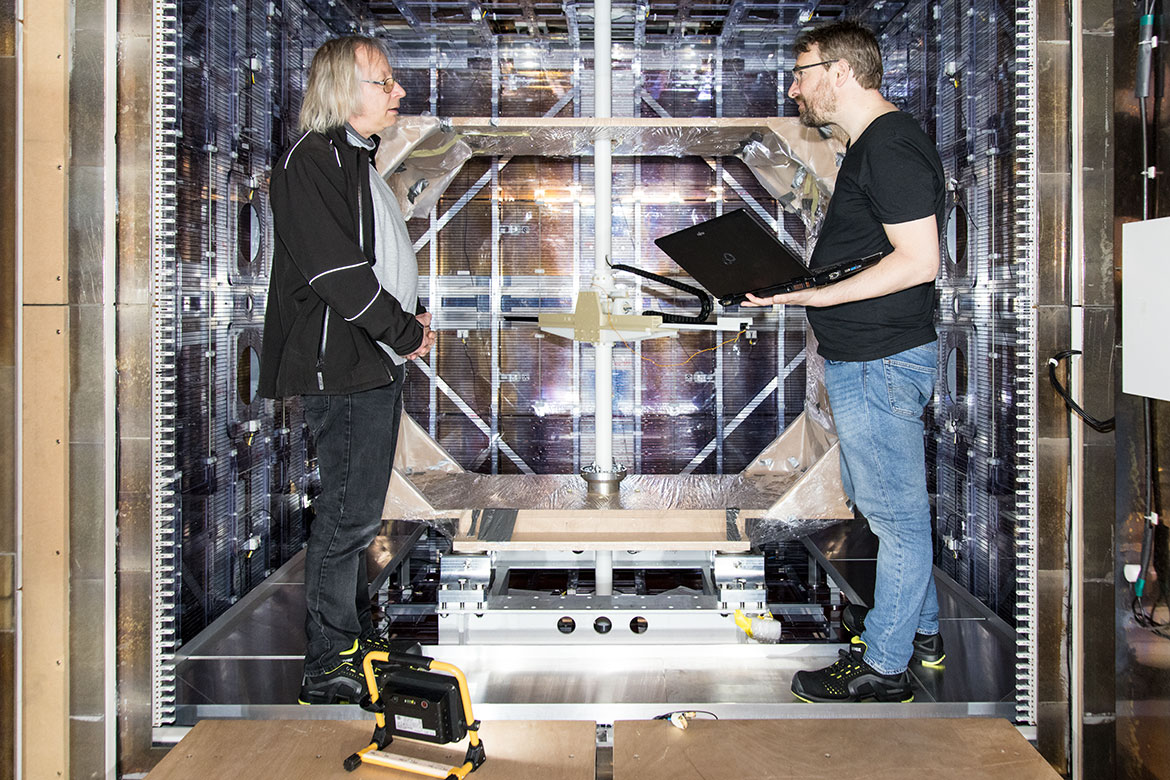
The researchers Bernhard Lauss and Georg Bison stand in the magnetically shielded chamber for ultracold neutrons. | Photo: Ruben Hollinger
A concentrated silence reigns in the large hall of the Paul Scherrer Institute (PSI). Outside, the wind lashes the spring rain into the faces of those passing by, but inside it’s warm and sheltered from the elements. An industrial crane glides almost silently across the room, bringing concrete elements weighing several tonnes towards workers who stack them neatly on top of each other as if they were Lego bricks. Hidden underneath them lies the heart of the PSI: its proton accelerator, which has been located here in Villigen in Canton Aargau since 1974.
“Here, you can see the large ring cyclotron”, says Klaus Kirch, the head of the Laboratory of Particle Physics at PSI, pointing from the gallery to a corner of the hall. The ring cyclotron is part of the proton accelerator, has a diameter of 15 metres, and is hidden under two metres of concrete that protects the researchers from radiation.
Kirch proceeds to explain how the cyclotron works. “Inside, protons are held in their orbit by eight magnets each weighing 240 tonnes. The protons are then accelerated to 80 percent of the speed of light”, he says. He uses a board to demonstrate how the proton beam is sent into ever larger orbits before being guided through the hall to the experimental facility, where various detectors are situated that are used for experiments by the Swiss research infrastructure for particle physics (CHRISP).
Of pions and muons
“There are two major trends in experimental particle physics”, says Kirch. “On the one hand, you can impart ever more energy to individual particles and try to create new particles through having them collide”. This is what happens, for example, at the Large Hadron Collider at CERN. But at PSI, Kirch and his team are pursuing a different approach. Here, they are generating especially intense particle beams. “We are using them to investigate rare processes and have to be able to measure them precisely”. Their goal is to find signals that cannot be explained by the Standard Model of particle physics. This Model describes three of the four fundamental forces known to us in the Universe, and classifies all known elementary particles.
The cyclotron is not in operation during our visit – it’s time for its maintenance. People everywhere are checking numbers and codes on their displays. Some are setting up cables to devices or configuring the experimental stations. Once everything is shielded again and reassembled, the ring cyclotron will once more start shooting out one of the most powerful proton beams in the world. After a slight left turn, the beam will collide in quick succession with two spinning graphite rings that project out into its path. “The impact of the proton beam on the atomic nuclei in the carbon target initially creates pions”, Kirch explains. “These particles comprise two quarks, and they decay into muons within a few fractions of a second”.
These elementary particles provide the basis for many experiments at CHRISP. They resemble electrons, but are some 200 times heavier and, at PSI, can be produced in large quantities. Their comparatively low speed enables unique experiments that might shed light on obscure aspects of the Standard Model.
Decaying trillions
After a short walk past control cabinets, up some stairs and across several galleries, we arrive at one of these experiments: MEG II. Behind an opaque plastic sheet, there is a long tube surrounded by cables, magnets and measuring devices. A researcher has begun climbing onto the detector to check the connections.
“At CHRISP, we are using MEG II to research into the decay of muons. We are looking for decay processes that ought not to exist in the Standard Model”, says Kirch. Muons have an extremely short lifetime. After about 2.2 microseconds, they decay into other, more stable particles – usually a positron and two neutrinos. In extremely rare cases, other decay paths might also be possible. However, no one has ever observed this. “We want to measure how likely it is for a muon to decay into a positron and a photon, in other words a light particle”. If they could observe such a process at PSI, it would be a sensation. “We are looking for something that no one has ever seen before. But with a beam as intense as the one here at PSI, we can increase the probability that we might be able to find it”.
In their previous experiment that ran at PSI from 2009 to 2013, they found that not even one out of 2.4 trillion muons was able to produce their sought-after result by decaying into a positron and a photon. This was the number of times they observed decaying muons without any such extraordinary event occurring. Despite everything, that experiment cannot be considered to have failed, because it changed the boundaries of what is theoretically possible, and remains informative for basic research.
Just a few metres away, a related experiment will soon be looking for another muon decay process that is also “actually forbidden” by the Standard Model. It’s called ‘Mu3e’ and will be used to measure whether muons can sometimes also decay into three electrons – two positively charged, one negatively. “Once this experiment is up and running, we will be able to observe one billion decaying muons every second”, says Kirch. That’s a lot of data that have to be reconstructed and assessed for purposes of later analysis. Today, we can also see a researcher working on Mu3e’s detector to ensure that it achieves such high-precision measurements. He is working right next to a dark-green metal cylinder, two metres in diameter, that will soon be used to encase the experiment.
Capturing ultracold neutrons
On the other side of the concrete wall of the hall, we find another CHRISP experiment. Here, the focus is not on muons, but on so-called ultracold neutrons – particles with extremely low energy levels. A so-called ‘kicker’ is used to divert the proton beam here from the main hall – rather like a track switch on the railways. Neutrons are then knocked out of atomic nuclei and slowed down so much that you could jog alongside them. “We can then capture them in containers and use them for a long-term experiment on the neutron electric dipole moment”, says Bernhard Lauss, who is leading the research project into the ultracold neutrons. The ‘neutron electric dipole moment’, or nEDM, measures how positive and negative electric charges are differently distributed in a body, hence CHRISP’s second large-scale experiment in this field is named: ‘n2EDM’.
According to today’s theory of elementary particles, neutrons ought not to have any measurable nEDM. This means there would be no charge differences inside the particles. “In highly simplified terms, n2EDM aims to determine whether the neutron spins a little when in an electric field”, says Lauss. “That could mean that this dipole moment really does exist after all”. If they could find this with their results, he believes it might then also be possible to explain better why matter exists in the universe. “According to the Standard Model, matter and antimatter ought to exist in roughly equal amounts”. But antimatter is nowhere to be seen.
In order to be able to measure this, they have to establish precisely controlled magnetic conditions. This is because the experiment is highly sensitive to the smallest shifts in magnetic fields. “If a truck drives past PSI, the magnetic field is altered”, says Georg Bison. He’s responsible for the shielding chamber, which is encased in a large wooden hut. On the inside, more than fifty kilometres of cable ensure that even the smallest magnetic fluctuations can be compensated for.
But in other respects, too, meticulous care must be taken to ensure that the neutrons can be measured undisturbed. The actual experiment takes place in a white cube situated in the wooden hut, whose sides contain several layers of a nickel-iron alloy. Its total weight is 45 tonnes. Three doors have to be opened into this mystical vault before you can get a glimpse inside. You also have to change your shoes or put on overshoes in order to ensure that no magnetic dust is carried into the experiment chamber. “One particle of magnetic dust in the wrong place, and the whole experiment is ruined”, says Bison.
Fourteen different research groups are involved in n2EDM. The magnetically shielded room is unique of its kind, and it alone cost some CHF 2.4 million – jointly funded by ETH Zurich, PSI and the Swiss National Science Foundation. As with their research into muons, Lauss and his colleagues are hunting for something that ought not to exist at all. And here, too, its much smaller, predecessor experiment produced no results. But Kirch, Lauss and Bison are not giving up. They’ll continue to do all they can to ensure that PSI can finally chalk up the sensational findings for which they’re hoping.